Alkene functionalization reactions are a staple of every undergraduate programme in chemistry and can be broadly divided into two families: (1) transformations that result in either temporary (for example, olefin metathesis) or permanent (for example, ozonolysis) C=C bond cleavage and (2) reactions resulting in products that maintain the original C–C σ-bond connectivity1,8. Among the latter, the broad family of 1,2-difunctionalization reactions9,10 ranges from classical halogenation to sophisticated transition-metal-catalysed processes11,12,13, and the nascent field of remote functionalization has emerged as a way of redirecting reactivity towards a distal position, away from the initial reactive site2,3,4,14,15,16,17,18,19,20. Indeed, a number of methods for alkene 1,3-difunctionalization using transition-metal catalysis have emerged in the literature. Such methods (Fig. 1a) invariably rely on a mechanistic handle to achieve regioselectivity: a directing group or ‘stopper’ (such as an arene ring that provides a benzylic resting point for the catalyst) must be embedded in the substrate, primarily because of the pronounced tendency of C(sp3)–M species to engage in β-hydride (β-H) elimination, resulting in the generation of Heck products5,6,7,21,22. Similarly, an extensive body of research on Friedel–Crafts-type reactions of alkenes is known (Fig. 1b)23. Primarily, reactions of acylium ions have been shown to afford the products of 1,2-difunctionalization, with product distribution often highly dependent on the nature of substrate, reagent and solvent (Fig. 1b, top). Intriguingly, Friedel–Crafts-type reactions of alkenes have also been found to enable remote functionalization of alkenes (Fig. 1b, bottom); such transformations, however, invariably suffer from undefined selectivity—often giving mixtures of 1,2- and 1,3-functionalization products24,25,26,27—or are possible only under substrate control or on specialized substrates28,29,30. Thus, although the corresponding reactivity, at its core that of the Friedel–Crafts reaction, has been explored, little is known about the factors governing the selectivity or predictability of such transformations, and the direct and general 1,3-difunctionalization of unactivated and unfunctionalized alkenes remains an unmet challenge.
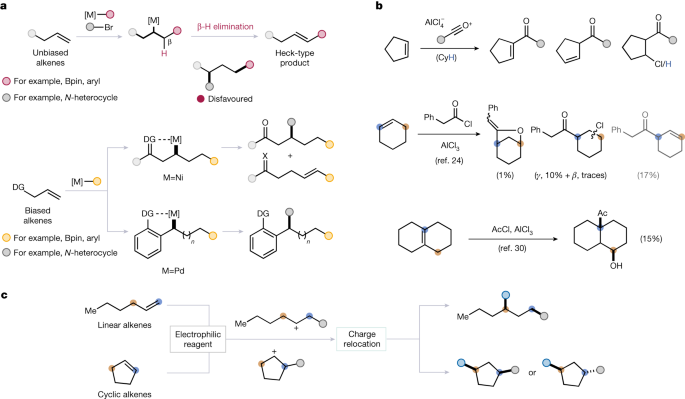
a, Current paradigm for the distal functionalization of alkenes, relying on directing-group-mediated metal catalysis. b, Friedel–Crafts-type reactions of alkenes generally provide mixtures of products—which depend on the substrate, reagent and solvent—with selectivity observed only with specialized substrates. c, This work: stereodivergent, reagent-controlled 1,3-difunctionalization of alkenes through charge relocation. DG, directing group; Bpin, pinacol boronate.
We present such a difunctionalization of unactivated alkenes under simple conditions, through which olefins are converted into products in which functionalization has occurred with generality at positions 1 and 3 (Fig. 1c). We achieved this by adapting electrophilic addition to alkenes as the basis for a general, predictable and highly selective strategy termed ‘charge relocation’—a synthetic logic in which incipient or localized charge at a given atom relocates to a defined position through a series of hydride shifts.
Well aware that classical electrophilic additions to double bonds hinge on immediate interception of an (incipient) positive charge by a nucleophile23, we became intrigued by the mode of electrophilic addition to a double bond in the absence of a suitable nucleophilic species. Over the course of these investigations we eventually found that the treatment of cyclohexene with an acylium cation carrying non-nucleophilic hexafluoroantimonate as the counter anion, generated in situ from acyl chloride and silver hexafluoroantimonate, swiftly and selectively formed a product of 1,3-hydroxyacylation following hydrolytic work-up (Fig. 2a; see Supplementary Information for additional conditions surveyed during optimization of reaction conditions). Notably, the success of the reaction was found to rely heavily on the nature of the halophilic reagent, with only silver hexafluoroantimonate providing a high yield of 19 whereas other silver salts or Lewis acids gave low levels of conversion to the desired product. Most interestingly, this reaction not only resulted in 1,3-difunctionalization but it did so with exclusive selectivity for the syn-products over a range of different unactivated alkenes, as depicted in Fig. 2.
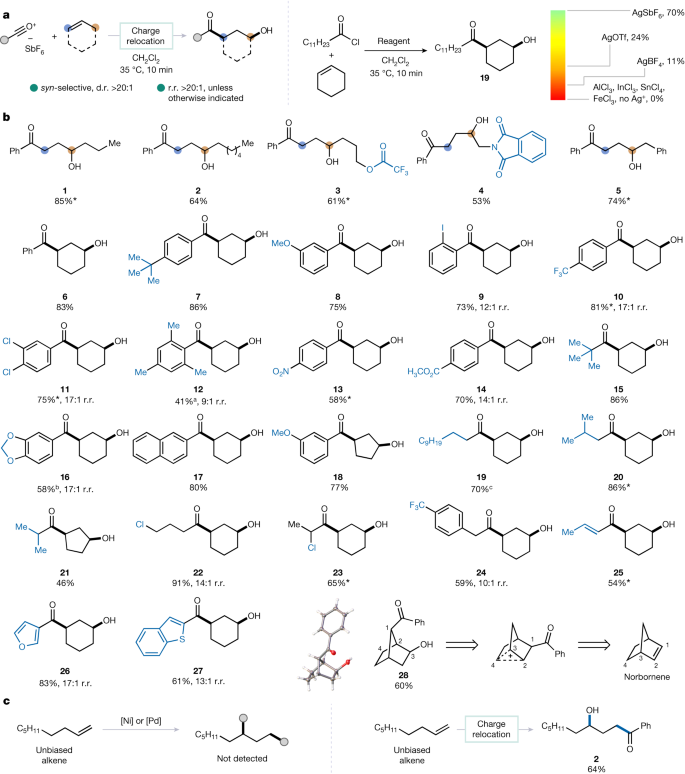
a, 1,3-Hydroacylation of cyclohexene. b, Scope of syn-alcohols. c, Comparison with transition-metal catalysis. All yields in the table of optimization correspond to nuclear magnetic resonance yields using 1,3,5-trimethoxybenzene as an internal standard. All products were obtained at greater than 20:1 d.r. and regioisomeric ratio (r.r.), unless otherwise mentioned. All yields correspond to isolated material. See Supplementary Information for further details and additional scope entries. *The reported yields correspond to averages over three runs. In cases in which competing pathways led to the observation of enone byproducts in the crude mixtures, the following percentages were observed: a37, b24, c30. Additional substrate scope is presented in Extended Data Fig. 1 and Supplementary Information.
Following this finding we turned to investigate the scope of this transformation (Fig. 2b), initially using a phenyl-substituted acylium ion. Our survey of linear alkenes, inherently lacking the ability to form diastereomeric products, showed high yields for a range of chain lengths (1 and 2). Appended functional groups were also found to be tolerated, with substrates bearing a trifluoroacetate (3) or phthalimide (4) providing the desired products at good yield. Importantly, 4-phenyl-1-butene—a substrate bearing potential bias due to the presence of a benzylic position—afforded exclusively the product 1,3-difunctionalization (5) with the benzylic site remaining untouched.
As highlighted above, the reaction with cyclohexene was found to deliver exclusively the product of syn-hydroxyacylation (6), a fact proven to be true for a large variety of arene-substituted acylium ions regardless of electronic or steric properties (7–17). The same stereochemical outcome was observed for a cyclopentene-derived ketoalcohol (18), as well as for products resulting from the addition of aliphatic acylium ions (19–24). Notably, an acryloyl-derived acylium ion also provided the desired product of syn-hydroxyacylation (25), as did those bearing heteroaromatic groups (26 and 27). Finally, when norbornene was employed, the intermediately formed, positively charged species underwent rearrangement (presumably via a non-classical carbocation), affording crystalline 28.
Figure 2c shows a comparison of this method with previously reported approaches to alkene 1,3-difunctionalization, relying on substrate rather than reagent control. Indeed, when a fully unbiased alkene (1-nonene) was subjected to established, transition-metal-catalysed protocols using either nickel or palladium catalysis, no products of 1,3-difunctionalization were obtained—rather, products of Heck coupling or non-specific decomposition were observed (see Supplementary Information for details on these reactions). By contrast, the protocol presented herein—as shown above—provided the product of 1,3-difunctionalization (2) at 64% isolated yield.
In subsequent investigations we found that, if the final work-up was preceded by treatment with dimethyl sulfoxide (DMSO)31, the corresponding products of anti-hydroxyacylation were obtained with excellent stereoselectivity (29–37, typically over 20:1 diastereomeric ratio (d.r.); Fig. 3), thus enabling flexible access to either isomer in a stereodivergent manner32,33,34,35,36,37. Replacement of the hydrolytic work-up by the addition of other nucleophilic sources such as chloride (38), bromide (39) or iodide (40) resulted in anti-halogenated products. Interestingly (Fig. 3), interception with amides such as N,N-dimethylacetamide or -formamide resulted in the isolation of anti-acyloxy-acylated products (41 and 42) whereas—even more intriguingly—the addition of the stable aminoxyl radical TEMPO provided anti-OTMP product 43 at high yield. Importantly, when the addition of DMSO was followed by treatment with triethylamine, the products of a 1,3-ketoacylation reaction were obtained (44–49). This is a process that, to the best of our knowledge, has no precedent in the literature and converts simple alkenes directly to 1,4-dicarbonyls37,38,39,40.
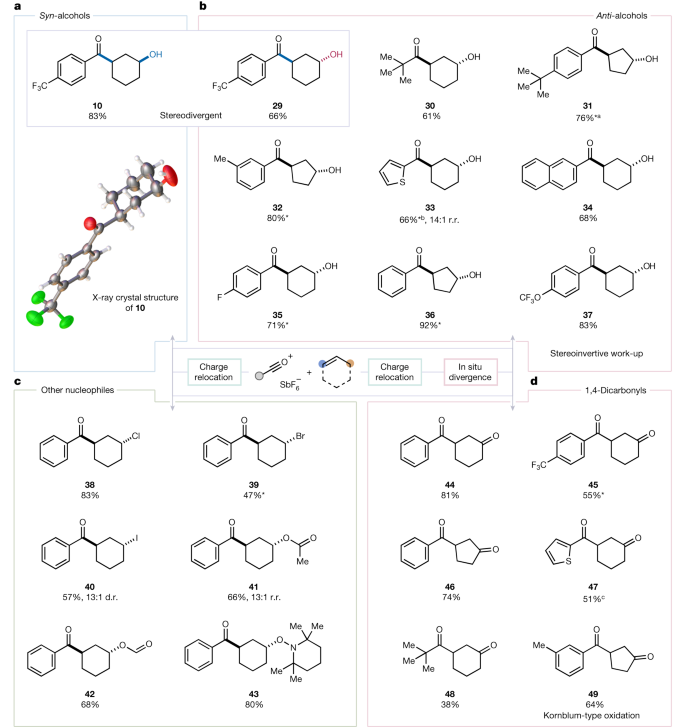
a, Selected example of syn-selective hydroxyacylation in contrast to anti-configured product 29. b, Scope of anti-selective 1,3-hydroxyacylation, achieved by the addition of DMSO at 35 °C and quenching with tetrabutylammonium bromide at room temperature. c, Additional 1,3-difunctionalization reactions using other nucleophiles—tetrabutylammonium halides, dimethylacetamide, dimethylformamide or TEMPO—at 35 °C. d, 1,4-Dicarbonyl synthesis through Kornblum-type oxidation, employing DMSO at 35 °C, followed by NEt3 at room temperature. All products were obtained at greater than 20:1 d.r. and 20:1 r.r., unless otherwise mentioned (Supplementary Information). *The reported yields correspond to averages over three runs. In cases in which competing pathways led to observation of enone byproducts in the crude mixtures, the following percentages were observed: a5, b10, c11.
Aiming to showcase the synthetic prowess of this facile, yet powerful, 1,3-alkene difunctionalization, we explored potential synthetic applications. We selected 4-ipomeanol (50), a model pulmonary pretoxin with activity for protein binding (N-acetyl cysteine and N-acetyl lysine)41,42, the reported synthesis of which is a multistep endeavour (Fig. 4a; five steps from diethyl furan-3,4-dicarboxylate)43. Alkene 1,3-difunctionalization, as presented here, instead enables the one-step synthesis of this compound (as well as the known phenyl-analogue 51 and other derivatives (52 and 53 (ref. 44)) from inexpensive 1-butene in a straightforward manner.
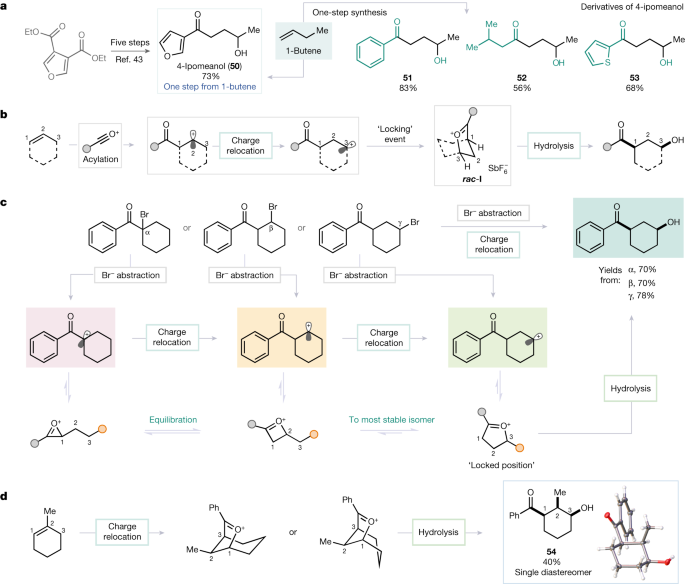
a, Synthesis of 4-ipomeanol and other biologically active molecules43. b, Proposed mechanism of 1,3-alkene difunctionalization, involving charge relocation. c, Mechanistic investigations support the hypothesis that nascency of the charge does not affect the constitution of the obtained product (Supplementary Information). d, Complete stereocontrol for the formation of a 1,2,3-trisubstituted cyclohexane.
From a mechanistic point of view (Fig. 4b) we believe that the 1,3-difunctionalizations presented above rely on a rapid isomerization event. This converts, under thermodynamic control, what would be the first intermediate of electrophilic addition23,24,27, the β-keto cation, into the rearranged, cyclic oxocarbenium ion rac-I45,46,47,48,49,50—with the formation of rac-I constituting a locking event to prevent further isomerization and non-selective product formation. This common intermediate is then intercepted either in hydrolytic fashion at the carbonyl (affording the syn-configured products) or through invertive displacement at the secondary sp3-centre C3 with other nucleophiles, resulting in the formation of the anti-configured products described above.
The concept of charge relocation is best illustrated by the mechanistic experiments depicted in Fig. 4c. We established that, regardless of where the carbocation is initially formed (by bromide abstraction), the overwhelming majority of the material is converted into the expected 1,3-difunctionalized target: the carbocation was drawn to the γ position independently of its nascent state.
The reactions of more complex substrates also proved interesting. When 1-methylcyclohexene, a trisubstituted alkene, was used, the all-syn-hydroxyketone 54 was obtained as a single diastereomer (see X-ray structure to the right; Fig. 4d). This stereochemical outcome is probably governed by the preferred equatorial orientation of the only substituent not bound within a ring, the methyl group (Fig. 4d).